Protein Structure Determination Techniques: X-ray Crystallography vs. Cryo-Electron Microscopy
Background Introduction Proteins are the fundamental molecules driving life processes, and their functions are predicated on their three-dimensional structures. These...
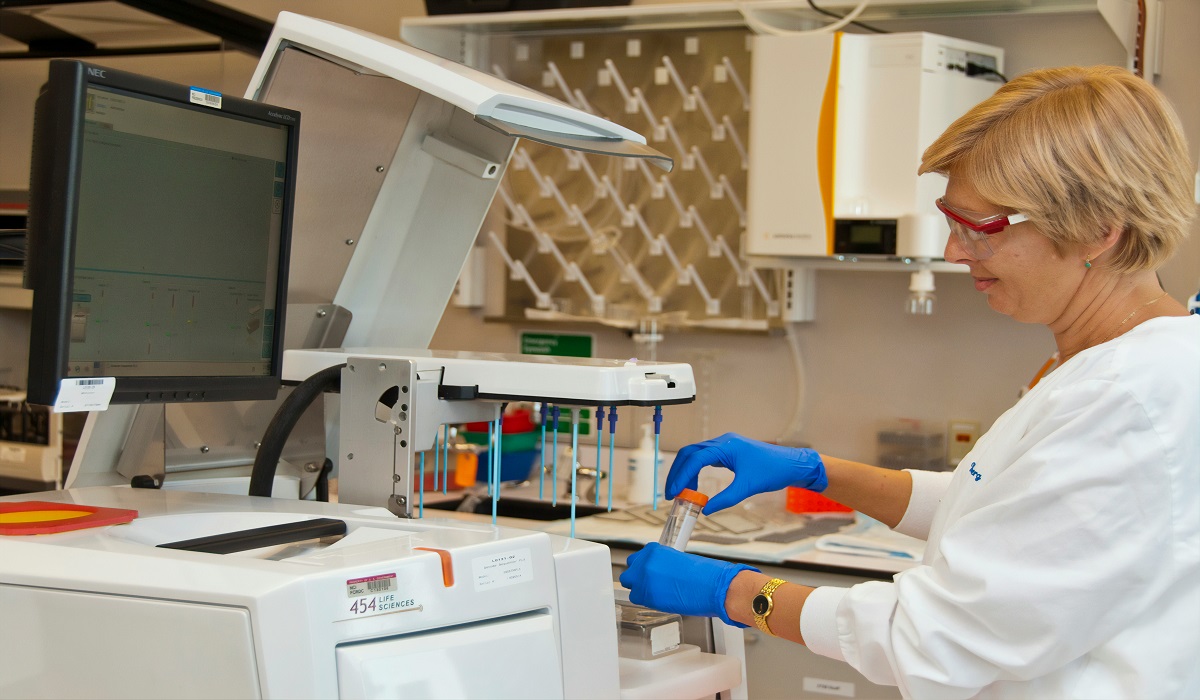
Background Introduction
Proteins are the fundamental molecules driving life processes, and their functions are predicated on their three-dimensional structures. These structures are characterized at four levels: primary structure (amino acid sequence), secondary structure (local conformations such as α-helices and β-sheets), tertiary structure (overall folding of a single polypeptide chain), and quaternary structure (assembly of multiple subunits).
For instance, the catalytic activity of enzymes is contingent on the precise three-dimensional configuration of their active sites, whereas the signal transduction function of membrane proteins is intricately linked to conformational shifts in their transmembrane domains. Studying protein structures not only uncovers mechanisms of action but also lays the groundwork for drug design, disease treatment, and synthetic biology. However, proteins exist on the nanometer scale, beyond the resolution of traditional optical microscopes, necessitating high-resolution techniques to resolve their atomic architectures.
X-ray crystallography and cryo-electron microscopy (Cryo-EM) are the leading techniques for protein structure determination.
X-ray Crystallography vs. Cryo-EM
X-ray Crystallography
The advent of X-ray crystallography in the early 20th century was pioneered by the Bragg father-son duo. They formulated Bragg’s Law, elucidating the quantitative relationship between X-rays and atomic arrangements in crystals, thus founding the framework for structural analysis.
The significance of X-ray crystallography is profound. By the close of the 20th century, it had been instrumental in 27 Nobel Prizes. A notable application was Rosalind Franklin’s 1953 work, which provided the first “Photograph 51” of the DNA B-form diffraction pattern, instrumental in suggesting DNA’s helical structure. Franklin’s image underpinned Watson and Crick’s double-helix DNA model, a cornerstone in molecular biology.
The discovery of myoglobin’s structure in 1958 using X-ray crystallography marked a major stride in protein structural analysis. The technique leverages the interaction of X-rays with atoms in protein crystals to produce diffraction patterns. Through analysis of these patterns combined with Fourier transforms, a three-dimensional electron density map emerges, from which an atomic model is deduced.
However, X-ray crystallography presents challenges: membrane protein crystallization and the phase problem. Crystallizing certain proteins, especially membrane-bound ones, is arduous. Innovations like synchrotron radiation have enhanced the applicability of X-ray crystallography by providing powerful, precise X-rays, crucial for resolving complex structures like GPCRs. Advanced techniques such as X-ray free-electron lasers (XFELs) and the utilization of cryo-cooling for crystals have expanded X-ray crystallography’s capability by allowing for synchronization of data collection with dynamic biological processes, thus facilitating the observation of transient states and intermediate structures.
Cryo-Electron Microscopy (Cryo-EM)
Cryo-EM experienced a transformative “resolution revolution” in the 2010s. Unlike X-ray crystallography, it bypasses crystallization, analyzing samples in solution, ideal for examining proteins with diverse conformational states.
Protein samples are swiftly frozen in liquid ethane at -196°C to form a vitreous ice that prevents structural ice crystal formation, preserving native states. Electrons, rather than X-rays, create high-resolution images, as hundreds of thousands of two-dimensional images are computationally recombined into a three-dimensional structure using advanced algorithms like single-particle analysis.
Cryo-EM’s flexibility and ability to capture large complexes in various conformations sets it apart. For example, it has elucidated structures like ribosomes and viral capsids at near-atomic resolutions. In COVID-19 research, it depicted spike protein conformations crucial for understanding viral entry mechanisms and vaccine development.
Innovations such as phase-plate technology have improved image contrast by manipulating the phase of electron waves, thus providing sharper images of biological specimens (Additional insights on hardware innovation in Cryo-EM were included). Direct electron detectors and deep learning-based software (like cryoSPARC) assist in refining image quality and analyzing vast data sets efficiently. However, Cryo-EM still demands high sample purity and homogeneity to achieve optimal results, particularly at resolutions comparable to X-ray crystallography.
Summary
X-ray crystallography and cryo-electron microscopy stand as foundational pillars in structural biology, each offering distinct benefits and challenges.
X-ray crystallography is exceptional for atomic-level resolution and is the go-to for smaller, rigid proteins that crystallize well. Conversely, Cryo-EM is suited to visualizing large asymmetric complexes and dynamic states, capturing a breadth of conformational changes.
Together, these techniques have vastly advanced our understanding of protein mechanisms. As integration with AI and computational approaches like AlphaFold progresses, the prediction and functional understanding of proteins accelerates, pushing structural biology to encompass more dynamic, system-wide analyses from the cellular to the molecular level. Creative Biostructure’s comprehensive Cryo-EM services integrate these technologies with state-of-the-art, AI-driven modeling and simulation tools, facilitating a deeper understanding of protein dynamics and interactions.
References
Ma, L. D. (2014). The Centennial Glory of X-ray Crystallography. Progress in Physics, 34(2), 47–117. doi: 10.13725/j.cnki.pip.2014.02.001
https://digitalpaper.stdaily.com/http_www.kjrb.com/kjrb/images/2014-03/30/02/DefPub2014033002.pdf
Palczewski, K., Kumasaka, T., Hori, T., Behnke, C. A., Motoshima, H., Fox, B. A., … & Miyano, M. (2000). Crystal structure of rhodopsin: A G protein-coupled receptor. Science, 289(5480), 739-745.
Rasmussen, S. G., Choi, H. J., Rosenbaum, D. M., Kobilka, T. S., Thian, F. S., Edwards, P. C., … & Kobilka, B. K. (2007). Crystal structure of the human β2 adrenergic G protein-coupled receptor. Nature, 450(7168), 383-387.
Rasmussen, S. G., DeVree, B. T., Zou, Y., Kruse, A. C., Chung, K. Y., Kobilka, T. S., … & Kobilka, B. K. (2011). Structure of a nanobody-stabilized active state of the β2 adrenoceptor. Nature, 477(7366), 549-555.
McCoy, A. J. (2007). Solving structures of protein complexes by molecular replacement with Phaser. Acta Crystallographica Section D: Biological Crystallography, 63(1), 32-41.
Li, Z. F., & Gao, N. (2018). Cryo-EM Method for High-Resolution Structure Determination: A Brief Introduction to 2017 Nobel Prize in Chemistry. University Chemistry, 33(1), 1-6.